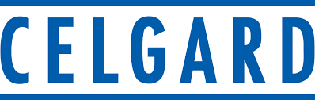
Celgard
Founded Year
1999About Celgard
Celgard is a company that focuses on the development and production of high-performance membrane technology, operating primarily in the energy storage and battery sectors. The company's main offerings include products used in a variety of energy storage applications, such as lithium-ion batteries, lithium primary batteries, and other specialty battery solutions, as well as in technical textiles and other barrier-type applications. Celgard primarily sells to sectors that require energy storage solutions and technical textiles, such as the electric vehicle industry and the medical personal protective equipment industry. It was founded in 1999 and is based in Charlotte, North Carolina.
Loading...
Loading...
Expert Collections containing Celgard
Expert Collections are analyst-curated lists that highlight the companies you need to know in the most important technology spaces.
Celgard is included in 1 Expert Collection, including Energy Storage.
Energy Storage
5,352 items
Companies in the Energy Storage space, including those developing and manufacturing energy storage solutions such as lithium-ion batteries, solid-state batteries, and related software for battery management.
Celgard Patents
Celgard has filed 185 patents.
The 3 most popular patent topics include:
- polymers
- rechargeable batteries
- membrane technology
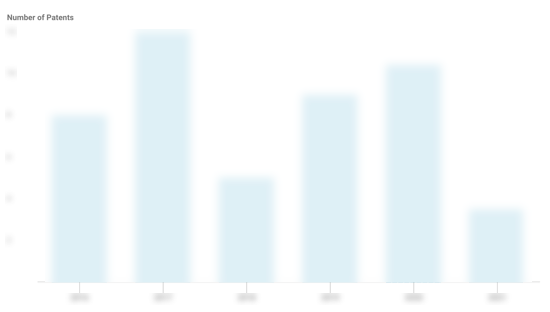
Application Date | Grant Date | Title | Related Topics | Status |
---|---|---|---|---|
3/2/2021 | 8/20/2024 | Membrane technology, Polymers, Geometrical optics, Semiconductor materials, Filters | Grant |
Application Date | 3/2/2021 |
---|---|
Grant Date | 8/20/2024 |
Title | |
Related Topics | Membrane technology, Polymers, Geometrical optics, Semiconductor materials, Filters |
Status | Grant |
Latest Celgard News
Apr 15, 2024
Abstract Engineering atom-scale sites are crucial to the mitigation of polysulfide shuttle, promotion of sulfur redox, and regulation of lithium deposition in lithium–sulfur batteries. Herein, a homonuclear copper dual-atom catalyst with a proximal distance of 3.5 Å is developed for lithium–sulfur batteries, wherein two adjacent copper atoms are linked by a pair of symmetrical chlorine bridge bonds. Benefiting from the proximal copper atoms and their unique coordination, the copper dual-atom catalyst with the increased active interface concentration synchronously guide the evolutions of sulfur and lithium species. Such a delicate design breaks through the activity limitation of mononuclear metal center and represents a catalyst concept for lithium–sulfur battery realm. Therefore, a remarkable areal capacity of 7.8 mA h cm−2 is achieved under the scenario of sulfur content of 60 wt.%, mass loading of 7.7 mg cm−2 and electrolyte dosage of 4.8 μL mg−1. Introduction The earth-abundant and eco-friendly sulfur presents a high theoretical specific capacity of 1675 mA h g−1, which in turn enables an ultrahigh theoretical energy density of 2600 Wh kg−1 for lithium–sulfur batteries (LSBs) 1 , 2 , 3 . In this sense, the rechargeable LSBs are considered as one of the most promising candidates for the next-generation renewable energy storage systems, especially in the context of carbon neutral goal 4 , 5 . Nevertheless, a multitude of obstacles have severely hindered their commercial application 6 , 7 . To begin with, the soluble lithium polysulfides (LiPSs) usually diffuse and shuttle between the cathode and anode, resulting in irreversible capacity fading 8 , 9 , 10 . Another detrimental concern lies in the sluggish redox reactions, which accordingly triggers LiPS accumulation and deteriorate sulfur utilization 11 , 12 . Beyond that, the uncontrolled growth of lithium dendrites on the anode side also gives rise to the battery performance degeneration and potential safety issues, which further worsen the energy density and duration of LSBs 13 , 14 . Recently, various electrocatalytic strategies (metal oxides 15 , 16 , sulfides 17 , selenides 18 , 19 , phosphides 20 , 21 and nitrides 22 ) have been proposed to combat the aforementioned issues, mainly pertaining to vacancy defects 23 , 24 , 25 , heteroatom doping 26 , 27 , 28 , and interface engineering 29 , 30 , 31 . Thereof, the introduction of over-weighted metal compounds inevitably degrades the overall energy density of the LSBs 32 , 33 . To this end, single-atom catalysts (SACs) with atomically dispersed sites anchored on suitable substrates can enable the maximum atomic utilization and robust catalytic activity 34 . To date, multifarious SACs supported by carbon substrates have emerged to enhance the electrocatalytic effect and promote the redox kinetics of sulfur cathodes 35 , 36 , 37 . In terms of the lithium anode, SACs also can afford lithiophilic sites to homogenize the lithium nucleation and thus suppress the growth of lithium dendrites 38 , 39 . Despite these great breakthroughs in LSBs, it remains a tremendous challenge to achieve high-efficiency, stable, and high-loaded SACs 40 , e.g., atomic termination 41 , dual-atom active metal sites 42 , 43 , and so on. Especially, for dual-atom approach, each active site can contain a pair atom for participating the adsorption or catalytic processes. For instance, the oxygen-bridged indium-nickel atomic pairs recently have been reported as dual-atom active sites to promote CO2 reduction 44 . However, heterogeneous molecule catalysts with atomic metal sites have been scarcely reported in LSB field to date 45 . Moreover, the function of binuclear metal centers in molecule catalysts can be conceived toward the optimal selection for breaking through the activity limitation of mononuclear metal center. Along this line, the dual-metal molecule catalysts are expected to inject new life into the catalyst investigation for LSBs and spark broad interests in the future. On the proof of this concept, we introduce chlorine (Cl) bridge bond-enabled molecularly dispersed Cu-based complexes as the electrocatalysts in LSBs and investigate the battery performance, involving discharge capacity (C), rate capability as well as cycling stability. In detail, the existing Cl bridge bonds lead to the higher activity of homonuclear dual Cu atom catalysts (Cu-2) for the evolutions of sulfur and lithium species than the mononuclear Cu atom catalyst (Cu-1) throughout the whole electrochemical reaction based on the various analysis, including operando Raman spectra, electrochemical detections, synchrotron radiation X-ray three-dimensional nano-computed tomography (X-ray 3D nano-CT), small angle neutron scattering (SANS) and theoretical simulations. Benefiting from the well-designed coordination environment, Cu-2 endows the LSB with a high discharge specific capacity of 1140.6 mA h g−1 at 0.2 C and a retention of 91.3% after 100 cycles. Even with a high sulfur loading of 7.7 mg cm−2, the S/Cu-2 cathode can obtain an areal capacity (CA) of 7.8 mA h cm−2, which propels the real implementation of highly efficient and remarkably durable LSBs. Results The 1-Cuphen and 2-Cuphen complexes are first synthesized by tuning the mole ratio of Cu2+ deriving from copper (II) chloride dihydrate and 1,10-phenanthroline (phen) ligand into 1:2 and 1:1, respectively (Fig. 1 ). The as-obtained Cu complexes (green powder) are loading on the surface of graphene substrate for the synthesizing the Cu-1 and Cu-2 powders (Figs. S1 , S2 ). Note that the large planar aromatic structures of Cu complex molecules can interact with the graphene by the formed π–π interaction. For such a PS2−-Li+ coupled LSB system, both of the Cu-2 and Cu-1 can make full use of the active Cu single atoms to effectively regulate Li–S electrochemical reactions. Of particular note, the optimized coordination environment of Cu-2 based on the Cl bridge bonds realizes more remarkable function than Cu-1 in synchronously manipulating the evolutions of sulfur and lithium species. The detailed working mechanism of Cu-2 and Cu-1 are illustrated in Fig. 2 . As depicted in Fig. 2a , the Cl bridge bonds give rise to the generation of the homonuclear Cu atoms in pairs for Cu-2. The homonuclear dual Cu centers and their unique coordination with N and Cl atoms endow the Cu-2 with the remarkable advantages in chemically anchoring and kinetically converting LiPSs in contrast to that of Cu-1. Whilst, Cu-2 affords more uniform and lithiophilic nucleation sites to guide the homogeneous Li deposition for effective dendrite suppression than Cu-1 (Fig. 2b ). Fig. 1: Schematically illustrating the designs of Cu-1 and Cu-2. Cu-1 is obtained by first tuning the mole ratio of Cu2+ and phen as 1:2 to gain 1-Cuphen and then dispersing the 1-Cuphen on the surface of graphene. The Cu-2 is achieved by first adjusting the mole ratio of Cu2+ and phen as 1:1 to attain 2-Cuphen, and subsequently dispersing it on the surface of graphene. a LiPS conversions catalyzed by Cu-1 and Cu-2. b Lithium evolutions manipulated by Cu-1 and Cu-2. Ultraviolet–visible (UV–vis) spectra were first collected to confirm the coordination of Cu2+ and phen. As displayed in Fig. 3a , the red shift of the characteristic peak located at ~297 nm can be attributed to the formed coordination of Cu2+ and phen. Besides, in the visible light region, a broad absorption band (~750 nm) is observed, substantiating the generated Cu2+-based complexes 46 . Fig. 3b displays the Fourier transform infrared spectroscopy (FTIR) spectra of phen, 1-Cuphen, and 2-Cuphen. The peaks at 1629 and 1519 cm−1 assigning to the stretching vibrations of C=N and C=C bonds in phen aromatic ring respectively shift to 1621 and 1508 cm−1 after coordinating with Cu2+ in 2-Cuphen. Similar red shift phenomenon can be also observed in the FTIR spectrum of 1-Cuphen. Note that the formation of Cu–N bonds in complexes tends to change the configuration, symmetry and electron density distribution of the whole ligand, hence leading to the shift of all the peaks. The atomic structures of 1-Cuphen and 2-Cuphen by single crystal X-ray diffraction (XRD) tests are shown in Fig. 3c , e (CCDC: 2217502 and 2047772). The detailed bond lengths and angels are displayed in Tables S1 and S2 . In Fig. 3c , two Cu atoms are bridged by two Cl atoms in 2-Cuphen molecule to form the symmetrical binuclear Cu sites. The spherical aberration corrected transmission electron microscope (TEM) images show the crumpled graphene morphologies of Cu-1 and Cu-2 (Figs. S3 , S4 ). High-angle annular dark-field scanning TEM (HAADF-STEM) images in Fig. 3d , f reveal that the Cu single atoms are uniformly distributed on the substrates for both Cu-2 and Cu-1. Furthermore, Cu atoms in Cu-2 are in pairs (marked with red circles), proving the formation of binuclear Cu sites. The distances between the proximal two Cu atoms in the HAADF-STEM image of Cu-2 are around 0.35 nm, in accordance with the results of single crystal XRD tests (Fig. S5a, b ). For the Cu-1 sample, the calculated distances between the neighboring two Cu atoms are obviously fluctuant (Fig. S5c , d ). The above results demonstrate that the Cl-bridge pairs maintain the structural stability of proximal two Cu atoms in Cu-2 sample. Inductively coupled plasma optical emission spectrometer (ICP-OES) tests were conducted to experimentally probe the content of Cu centers in Cu-1 and Cu-2. According to the measurement results, the Cu contents in Cu-1 and Cu-2 are 1.16 and 1.18 wt%, respectively, substantiating the similar contents of Cu centers in the two catalysts. Fig. 3: Morphological and structure characterizations of electrocatalysts. a UV–vis and b FTIR spectra of phen, 1-Cuphen and 2-Cuphen. c, e Molecular structure of 2-Cuphen and 1-Cuphen. d, f Atomic-resolution HAADF-STEM images of Cu-2 and Cu-1. g, h Experimental and simulated XRD patterns of 2-Cuphen and 1-Cuphen. i, j TEM elemental mapping images of Cu-2 and Cu-1. k Cu 2p XPS spectra of Cu-2 and Cu-1. The phase structures of 1-Cuphen and 2-Cuphen by powder XRD measurements are in line with the simulated data stemming from the single crystal XRD defined results (Fig. 3g , h ). In comparison, there is no characteristic peaks of 1-Cuphen and 2-Cuphen existing in the XRD patterns of Cu-1 and Cu-2, respectively, indicating the molecule dispersion of 1-Cuphen and 2-Cuphen on graphene (Fig. S6 ). The scanning TEM (STEM) images of samples are displayed in Fig. S7 . And the elemental maps of Cu, N, Cl, and C demonstrate that the 1-Cuphen and 2-Cuphen molecules are homogeneously distributed onto the graphene substrates (Fig. 3i , j ). X-ray photoelectron spectroscopy (XPS) was utilized to explore the chemical compositions of the two electrocatalysts (Figs. 3k and S8 – 10 ). It can be clearly witnessed the signals of bridged and nonbridged Cl atoms in Cu-2 with the corresponding binding energy as 198.2 and 197.8 eV for Cl 2p3/2, respectively, which is quite different with those for Cu-1 (Fig. S8 ). The Cu 2p spectrum of Cu-2 displays a lower binding energy of 934.8 eV than Cu-1 (934.9 eV), indicative of their different coordination environments. Finally, the N2 adsorption-desorption isotherms were applied to obtain the Brunauer–Emmett–Teller (BET) surface areas of samples. And the BET surface area values of phen loaded graphene (rphenGO), Cu-1, and Cu-2 are 91.0, 103.4, and 93.8 m2 g−1, respectively (Fig. S11 ). LiPS adsorption capability is the prerequisite for the shuttle effect alleviation. In this case, visualized Li2S4 adsorption experiments were first executed to probe the adsorption properties of Cu-2 and Cu-1. As observed in Fig. S12 , the Li2S4 solutions in vials with rphenGO and Cu-1 as the adsorbers turn light yellow after 10 h. As a contrast, Cu-2 almost decolor the Li2S4 solution after 10 h, indicative of its stronger LiPS adsorption capability. According to the UV–vis spectra, the absorption signal of Li2S4 at ~420 nm disappears due to the superior Cu-2 capture capability in the sharp contrast with the scenarios of rphenGO and Cu-1 samples 47 . To further probe the affinity of Li2S4 by Cu-1 and Cu-2, XPS was employed to inspect the chemical compositions of samples after the visualized adsorption measurements. As displayed in Fig. S13 , new peaks of Cu 2p3/2 are detected at lower binding energies for Cu-2 (932.4 eV) and Cu-1 (932.5 eV), indicating that Cu atoms can serve as the active centers for anchoring LiPSs. More importantly, the peaks of Cu 2p3/2 shift by 2.4 eV and the new peak percentage are around 60% for both Cu-1 and Cu-2. Note that only one new peak appears in the Cu 2p3/2 spectrum of Cu-2, manifesting that the dual Cu atoms in Cu-2 act as double-adsorption sites, rather than a one center for anchoring Li2S4 species. The spectrum of S 2p shows peaks centered at 162.2 and 162.4 eV which can be assigned to 2p3/2 of Cu–S bond, further confirming the effective anchoring effect of Cu-2 and Cu-1 with Li2S4. Operando Raman tests were employed to track the evolution behavior of sulfur species in a real-time manner. The cathode was obtained by the disconnected coverage of slurry on a piece of Al mesh with a pore size of 0.5 mm × 0.1 mm. After drying, the cathode was cut into small disks with a diameter of 13.0 mm for the operando Raman cell fabrication. Note that the disconnected material coverage simultaneously provides the cathode and catholyte regions for the operando Raman signal collection. Figure 4a displays the operando Raman spectra of cathode and catholyte regions on separator throughout the complete discharge/charge procedure at 0.2 C. With respect to the cathode region, the peaks at ~219 and ~473 cm–1 are assigned to octatomic S8, while the sharp peak at ~153 cm–1 is ascribed to S8−2 48 , 49 . These Raman signals of S8 and S8−2 gradually decrease in the discharge procedure and then increase in the charge process, reflecting the dynamic changes of sulfur species on the surface of Cu-2. The Raman signals of soluble LiPSs were also tracked in the catholyte region. In detail, the signals of S4−2 (~202 cm−1), S6−2 (~327 and ~399 cm−1) and S8−2 (~279 cm−1) presents the same variation trend due to the management of Cu-2 during the discharge/charge process. Operando Raman spectra of catholyte regions with respects to Cu-1 and rphenGO were also shown in Fig. S14 . The catholyte region of S/Cu-2 shows the weaker soluble LiPS Raman signals along with the discharge proceeding in contrast to the other two samples. Particularly, the Raman signals of LiPSs in catholyte region of S/Cu-2 almost disappear at the end of discharge. These results further confirm the stronger LiPS anchoring ability of Cu-2 50 , 51 . The operando Raman test results reveal the effective manipulation of the dynamic evolution behaviors of LiPSs by Cu-2, implying the favorable functions of Cu-2 on the shuttle effect mitigation and redox reaction catalysis. Theoretical simulations based on density functional theory were performed to verify the interaction between complexes and sulfur species. In Fig. 4b , the binding energies between Cu-2 and Li2S6, Li2S4 and Li2S are 1.07, 1.21, and 3.04 eV, respectively, the values of which are much higher than those of Cu-1 (0.87, 1.02, and 2.40 eV) and rphenGO (0.42, 0.37 and 1.65 eV), substantiating the strong LiPS capture capability of Cu-2. The optimal adsorption configurations and other binding energy values for the three samples are displayed in Figs. S15 – 17 . The evolution pathways of sulfur species on the electrocatalyst substrates and the related Gibbs free energy values are exhibited in Figs. 4c and S18 . Notably, the negative Gibbs free energy change (∆G) for the conversion of solid S8 to liquid Li2S8 represents the thermodynamically spontaneous exothermic step. For the Cu-2, Cu-1, and rphenGO, the ∆G values for the rate-determining step are 0.38, 0.52, and 0.75 eV, respectively, indicating the more easily-occurred sulfur species reduction on Cu-2 52 . Fig. 4: Electrochemical tests, spectrum characterizations and calculation simulations of sulfur species evolution on various electrocatalysts. a Operando Raman spectra of S/Cu-2 cathode and catholyte on separator during the discharge/charge process at 0.2 C. b Binding energies of sulfur species on various substrates. c Gibbs free energy profiles for the sulfide conversion reactions on Cu-2 and Cu-1. d Potentiostatic discharge curves of Li2S8/tetraglyme solution on Cu-2, Cu-1 and rphenGO substrates, respectively. e SEM images of deposited Li2S. f The dissociation curves of Li2S on Cu-2, Cu-1 and rphenGO substrates. g SEM images of electrochemically dissociated Li2S. Chronoamperometry measurements were first conducted to evaluate the kinetics of Li2S precipitation and dissociation reactions on the surface of catalyst substrates. As depicted in Fig. 4d , the capacity of Li2S precipitation on Cu-2 at 2.05 V is 242.8 mA h gs−1, the value of which is much higher in contrast to that on Cu-1 (155.3 mA h gs−1) and rphenGO (131.4 mA h gs−1), implying the remarkable electrocatalytic activity of Cu-2 for the Li2S nucleation reaction. The cells were disassembled after the nucleation procedure to examine the morphology and distribution of Li2S on substrates. It can be witnessed in Fig. 4e that the Cu-2 substrate fulfills the most complete coverage and homogeneous deposition of Li2S among the three samples, further demonstrating the effectively propelled Li2S nucleation and growth kinetics on Cu-2. In addition, the decomposition profiles of Li2S on substrates were recorded by a potentiostatic charging the cells at 2.35 V. As shown in Fig. 4f , the capacities of Li2S dissociation on Cu-2, Cu-1, and rphenGO are 437.1, 371.3 and 325.5 mA h gs−1, respectively. Whilst, scanning electron microscopy (SEM) images of electrode after the dissociation reaction exhibit the cleaner surface than other two samples (Fig. 4g ). Note that the higher activity for the Li–S redox reactions is harvested by Cu-2 than other samples owing to the identified homonuclear dual Cu centers according to the above potentiostatic test results. The proximal binuclear Cu centers in Cu-2 as well as their optimized coordination environment provide more active interfaces for realizing the appropriate electrokinetic control of the Li2S nucleation and decomposition reactions, manifesting the kinetically promoted discharge and charge processes of the practically operated LSBs. Cyclic voltammetry (CV) tests were further performed to probe the catalytic conversion process of LiPSs based on various catalysts. Figure 5a manifests the CV profiles of symmetrical cells within the voltage window of –1 to 1 V at a scan rate of 50 mV s−1. It’s apparent that CP/Cu-2 shows higher redox peak current than CP/Cu-1 and CP/rphenGO, suggesting the enhanced catalytic activity of Cu-2 toward LiPS redox reactions 38 . With a lowered sweeping rate of 0.5 mV s−1, two pairs of reversible redox peaks of the CV curves in Fig. S19 are labeled as a, b, c, and d. Peak a and d stand for the reduction conversion of soluble LiPSs to Li2S. Peak b and c respectively represent the reversible procedure of peak d and a 14 . It can be clearly observed that the CP/Cu-2 exhibits the smaller polarization compared to CP/Cu-1 for the whole sulfur redox reaction. Linear sweep voltammetry (LSV) measurements were also carried out with S/Cu-2, S/Cu-1 or S/rphenGO as the cathode and lithium foil as the anode to gather the Tafel slope values. As shown in Fig. S20 , there are two clear reduction peaks at 2.2–2.4 V (peak I) and 1.9–2.1 V (peak II) in the LSV curves, corresponding to the reduction of S8 to soluble Li2Sn (4 ≤ n ≤ 8) and the subsequent conversion to insoluble Li2S2/Li2S, respectively. For S/Cu-2, the positive shift of the peak I and II in the LSV curve reveals the facilitated sulfur reduction process in contrast to the other two cathodes. Moreover, the fitting Tafel slope values of S/Cu-2 for peak I and II are 44.0 and 88.3 mV dec−1, respectively, the values of which are lower than those of S/Cu-1 (56.2 and 115.0 mV dec−1) and rphenGO (61.9 and 118.8 mV dec−1), implying more favorable conversions on Cu-2 (Fig. 5b , c ) 53 . The activation energy (Ea) for each conversion reaction step of sulfur intermediates to further reflect the catalytic activity of Cu-2. Electrochemical impedance spectra (EIS) tests under different temperatures were performed at various voltages where critical sulfur reactions occur (Fig. S21 ). Particularly, Nyquist plots at 2.4 and 2.1 V respectively represent the conversion step of soluble LiPSs and Li2S4 to Li2S2/Li2S. After fitting the circuits, Arrhenius equation was applied to calculate the values of Ea (Fig. S22 ). As displayed in Fig. S22d , the S/Cu-2 cathode obtains the lowest the values of Ea for each voltage among the three samples, further corroborating the high catalytic activity of Cu-2 for the whole sulfur conversion reaction. Fig. 5: The electrocatalytic activity analysis of electrocatalysts for sulfur conversion reactions. a CV profiles of Cu-2, Cu-1 and rphenGO based symmetric cells. b, c LSV curves with corresponding Tafel plots showing in the insets. d Schematic diagram of X-ray 3D nano-CT facility for sample observation. e X-ray 3D nano-CT images of Li2S precipitations on Cu-2, Cu-1 and rphenGO substrates. f Statistic volume ratios of Li2S deposits on various substrates based on X-ray 3D nano-CT analysis. Apart from the information from the surface of cathodes, the inner of cathodes more truly reflect the situations of Li2S nucleation reactions. Synchrotron radiation X-ray 3D nano-CT as a powerful imaging facility was employed to accurately evaluate the information of Li2S deposit in the inner of cathodes 54 . The catalyst/Li2S samples were acquired from the disassembled cells after the Li2S nucleation measurements. The 2D tomograms of samples based on various rotation angles were reconstructed to produce the final 3D visualized images (Fig. 5d ). According to the imaging results in Figs. 5e and S23 , the Li2S precipitations on Cu-2 exhibits more homogeneous spatial distribution than that on Cu-1 and rphenGO. The quantitative results of Li2S deposit on catalysts are displayed in Fig. 5f . As seen in Fig. 5f , the volume ratio of Li2S and Cu-2 is 26.4%, which is much larger than that on Cu-1 (18.7%) and rphenGO (11.9%), in accordance with the results of chronoamperometry measurements at 2.05 V. The synchrotron radiation 3D X-ray nano-CT results disclose that Cu-2 produces superior electrocatalytic effect on the whole cathode involving the surface and inner, which is beneficial to attaining the favorable electrochemical performance. These results confirm the proximal distance of Cu active sites and well-designed coordination structure of Cu-2 which enable the superior efficiency of Li2S conversion reaction on Cu-2. The lithium stripping and plating behaviors were explored by fabricating the Li//Li symmetric cells with catalyst coated on the commercial Celgard separator (Fig. S24 ). As depicted in Figs. 6a and S25 , at a current density of 1.0 mA cm−2 and a capacity of 1.0 mA h cm−2, the Li//Li symmetric cell inserted with bare commercial PP separator presents dramatic voltage fluctuations due to the uneven Li deposition and severe dendrite growth. As a contrast, Cu-2 electrocatalyst endows the cell with stable lithium plating/stripping overpotentials and prolonged cycling lifespan over 1100 h, surpassing the Cu-1 coated and pristine Celgard separator. Moreover, Li//Li symmetric cell with Cu-2 remains a stable voltage hysteresis of 11 mV, which is slightly lower than that of Cu-1 (inset of Fig. 6a ). The EIS of Li//Li symmetric cells were collected after cycling at 1.0 mA cm−2. The charge transfer resistance of cycled cell with Cu-2 is less than ~5.0 ohm, in sharp contrast to the ~70.0 ohm of the cell inserted with commercial PP separator (Fig. 6b ). Figure S26 illustrates the rate performance of the Li//Li symmetric cells with various separators under the current density varying from 1 to 3 mA cm−2 with a fixed capacity of 1 mA h cm−2. In comparison with other separators, Cu-2 coated separator enables the Li//Li symmetric cell to exhibit stable operation at different current densities. Although Cu-1 coated separator boosts the rate cycling performance of Li//Li symmetric cell than the commercial Celgard separator, short circuit phenomenon still appears under a high current density of 3 mA cm−2. The superior cycling stability and rate performance are harvested by the Li//Li symmetric cell inserted with Cu-2 coated separator, verifying that the binuclear Cu sites are conduced to forming stable solid electrolyte interface (SEI) film and homogenizing the lithium metal deposition. Fig. 6: Effect of electrocatalysts on lithium anodes. a Cycling performance of Li//Li symmetric cell with the Cu-1,Cu-2 coated separators and pristine separator at 1.0 mA cm−2. b EIS curves for Li//Li symmetric battery after cycling. c CE of Cu//Li cells with different separators at 1 mA cm−2. d Li 1s XPS fitting data with etching for cycled sample. e LiF content statistics from Li 1s XPS fitting data. f–h SANS matrix of scattering intensity signals and fitting curves from Li anodes in Li//Li symmetric cells with Cu-2, Cu-1, and PP separators after cycling. COMSOL results of Li plating with PP (i) and Cu-2 (j) coated separators. Coulombic efficiency (CE) is an important parameter to evaluate the reversibility of lithium plating/striping. Along this line, the CE values were collected by assembling Cu//Li cells with Cu severing as the working electrode and Li metal as the counter electrode. As shown in Fig. 6c , the asymmetric cell inserted with Cu-2 coated separator still obtains a CE value of 98.9% after 150 cycles, while the CE value for Cu-1-enabled cell is only 97.6% during 113 cycles. Pristine commercial separator only maintains stable operation for 70 cycles with the CE value as 96.7%. The lower CE may be attributed to the uneven and loose SEI film which is easily penetrated by the dendrites. Figure S27 exhibits the lithium plating curves of Cu//Li cells at the first cycle where the nucleation overpotentials, pertaining to the gap between the voltage dip and the voltage plateau, are quite different. Apparently, Cu-2 endows the cell with a nucleation overpotential of 31.7 mV, the value of which is lower than Cu-1 coated (34.1 mV) and pristine separator (87.1 mV) at 1 mA cm−2. To uncover the underlying mechanism for the superior lithium protection effect of Cu-2, the surface chemical compositions of lithium foil after cycling were investigated by XPS (Figs. 6d and S28 ). The peaks located at 56.3, 55.6, and 53.7 eV in Li 1s spectra are ascribed to LiF, Li2CO3 and Li2O, respectively. In addition, the peak at 54.5 eV is assigned to Li2O2 or LiOH which are unstable ingredients for SEI film 55 . Figs. 6e and S29 further demonstrate the total contents of LiF, Li2O2, Li2O and LiOH analyzed by the Li 1s XPS fitting data. The higher LiF ratio (30.3%) manifests the more stable SEI film in the Cu-2-enabled cell as compared to that of Cu-1 coated (14.0%) and pristine PP separator (4.1%). The structures of lithium anodes after stripping and plating procedures were further detected by SANS and SEM. The cavity stemming from the nonuniform lithium deposition at anode has a trend to display strong scattering signals. The scattering intensity I(Q) to scatter vector Q plots are fitting in SASfit software with model independent analysis involving Guinier and Porod approximations. And the gyration radius (Rg) can be calculated from the scattering curves. The value of Rg is in the order of Cu-2 < Cu-1 < PP, which suggests that the Cu-2 separator enables more dense lithium deposition than Cu-2 and PP. The results are in accordance with the depositing morphologies detected by SEM (Fig. 6f–h , insets). As expected, the surface of lithium foil is uneven, angular and loose for the cell inserted with pristine PP separator. On the contrary, the morphology of lithium foil with regard to the cell inserted with Cu-2 coated separator is more smooth and dense, further corroborating the effective lithium dendrite inhibition by Cu-2. Furthermore, the morphology of anode and the concentration of electrolyte during the deposition process were calculated based on finite element analysis (Fig. 6i , j ). The results show Cu-2 endows the anode with a relatively dense deposition without obvious dendrite formation, indicating its better dendrite suppression ability. By contrast, the concentration gradient of lithium ions for initial sample and Cu-1 sample (Fig. S30 ) are smaller, and the dendrites are much serious. These results substantiate that the Cl bridge bond-enabled Cu-2 acts as favorable regulator in lithium stripping and plating procedures. The coin-type LSBs with S/Cu-2, S/Cu-1, S/rphenGO, and S/rGO cathodes were respectively assembled to test their electrochemical performance. The rate capacities were first measured and the results are shown in Fig. 7a . At 0.2, 0.5, 1.0, and 2.0 C, the S/Cu-2 cathode delivers reversible capacities of 1121.1, 1028.8, 936.4, 808.6 mA h g−1, respectively. When the discharge current is switched back to 0.2 C, a high reversible discharge capacity of 1100.1 mA h g−1 is recovered, indicative of the rate capability of S/Cu-2 cathode. For the S/Cu-1 cathode, the discharge capacities are 1100.2, 959.6, 853.6, and 755.8 mA h g−1 in the same rate order and the recovered capacity at 0.2 C is 981.7 mA h g−1. The S/rphenGO delivers the capacities of 1034.3, 737.9, 554.4, and 400.3 mA h g−1 and the S/rGO presents the lowest rate capacities (819.3, 574.1, 334.9, and 200.0 mA h g−1) at 0.2, 0.5, 1.0 and 2.0 C. Moreover, the reaction polarization is assessed by the overpotential calculated by the voltage gap from the charge/discharge profiles (Figs. 7b and S31 ). The lowest voltage gaps at various rates of S/Cu-2 among all the samples further manifest the effectively regulated polarization by Cu-2. The cycling performance of cathodes at 0.2 C was evaluated. In Figs. 7c–e and S32 , the S/Cu-2 cathode delivers a higher initial capacity of 1140.6 mA h g−1 and a more remarkable capacity retention of 91.3% over 100 cycles in contrast with those of S/Cu-1 (1062.0 mA h g−1 and 80.0%), S/rphenGO (1049.3 mA h g−1 and 71.1%) and S/rGO (802.7 mA h g−1 and 74.7%), validating its favorable cycling performance. The cathodes after 100 cycles at 0.2 C were inspected by SEM. It can be seen that both the S/Cu-2 and S/Cu-1 cathode maintain better structural integrity and robustness while obvious cracks exist in the S/rphenGO and S/rGO cathode (Fig. S33 ). Furthermore, the S/Cu-2 cathode holds the capacity retentions of 91.1%, 84.2%, 77.1%, and 69.6%, respectively, when working at 100, 200, 300, and 400 cycles, corroborating the remarkable optimization impact of binuclear Cu centers in Cu-2 on the evolution behaviors of sulfur and lithium species (Fig. 7f ). Fig. 7: Electrochemical performance of LSBs with various cathodes. a Rate capabilities of S/Cu-1, S/Cu-2, S/rphenGO and S/rGO cathodes. b Voltage gaps of S/Cu-1, S/Cu-2, S/rphenGO and S/rGO cathodes at various rates. c Cycling stability of the cathodes at 0.2 C. d, e Galvanostatic discharge-charge curves of different cycles at 0.2 C for S/Cu-2 and S/Cu-1. f Cycling performance of S/Cu-2 cathode at 2 C. g Cycling performance of the S/Cu-2 cathode with the high-loading sulfur. h High-sulfur-loading LSB performance comparisons between this work and other reports. i Schematic diagram of S/Cu-2-enabled pouch cell. j Long-term cycling performance of the S/Cu-2 cathode at 0.2 C for pouch cell. Authors and Affiliations State Key Laboratory of Environment-Friendly Energy Materials, School of Materials and Chemistry, Tianfu Institute of Research and Innovation, Southwest University of Science and Technology, Mianyang, 621010, China Qin Yang, Lixian Song & Yingze Song Department of Chemistry, University of Science and Technology of China, Hefei, 230026, China Jinyan Cai & Gongming Wang Key Laboratory of Automobile Materials MOE, School of Materials Science & Engineering, Jilin Provincial International Cooperation Key Laboratory of High-Efficiency Clean Energy Materials, Jilin University, Changchun, 130012, China Guanwu Li, Dong Wang & Weitao Zheng Tsinghua-Berkeley Shenzhen Institute & Tsinghua Shenzhen International Graduate School, Tsinghua University Shenzhen, Shenzhen, 518055, China Runhua Gao, Zhiyuan Han & Guangmin Zhou Key Laboratory of Neutron Physics and Institute of Nuclear Physics and Chemistry, China Academy of Engineering Physics, Mianyang, 621999, China Jingjing Han & Dong Liu Materials Science and Engineering, Physical Science and Engineering Division, King Abdullah University of Science and Technology (KAUST), Thuwal, 23955-6900, Saudi Arabia Zixiong Shi Contributions Y.S. and G.Z. conceived and designed the experiments. Q.Y. prepared the electrode materials and conducted the characterizations of materials and LSBs. J.C. performed the theoretical simulations with the G.W.’s guidance. G.L. carried out the finite element simulations with the guidance of D.W. and W.Z., R.G., Z.H. and Z.S. helped to analyze the working mechanism of catalysts. J.H. performed the SANS tests with D.L.’s guidance. L.S. conducted the synchrotron radiation X-ray 3D nano-CT tests. Q.Y. and Y.S. were mainly responsible for preparing the manuscript with input from all other authors. All authors discussed the results and commented on the manuscript. All authors have given approval to the final version of the manuscript. Corresponding authors
Celgard Frequently Asked Questions (FAQ)
When was Celgard founded?
Celgard was founded in 1999.
Where is Celgard's headquarters?
Celgard's headquarters is located at 13800 South Lakes Drive, Charlotte.
Who are Celgard's competitors?
Competitors of Celgard include Natron Energy and 8 more.
Loading...
Compare Celgard to Competitors
FlexEl is a green-tech company developing high performance, flexible power and flexible powered device applications. A technology spin-out from the University of Maryland, FlexEl works with partner companies to create, design, develop, license and/or manufacture flexible electronics and power management systems. FlexEl has developed custom BatteryCloth for applications including high performance, wearable electronics, remote sensing and transmission, military applications, and consumer product devices. FlexEl's core technologies (i.e., battery and energy harvesting technologies) emanate from the company's original work on high capacity power solutions for wireless sensor networks and active RFID tags.
Infinite Power Solutions is a company focused on energy and technology within the electronics and equipment industry. The company offers reviews and information on a wide range of products and technologies, including solar power, energy storage, computers, electronics, smart devices, appliances, satellites, and rechargeables. It primarily serves individuals and businesses interested in the latest advances in energy, technology, and power. It was founded in 2001 and is based in Littleton, Colorado.

BrightVolt is a company focused on the development of advanced battery technology, operating within the energy and technology sectors. The company's main offerings include rechargeable solid-state batteries, which are used to power a variety of applications such as hybrid and electric vehicles, consumer electronics, medical and wearable devices, and other information technology products. BrightVolt primarily sells to the automotive, industrial, medical, wearable technology, and consumer products sectors. BrightVolt was formerly known as Solicore Inc. It was founded in 2001 and is based in Redmond, Washington.
Blue Spark focuses on the development of sign-monitoring solutions in the healthcare sector. The company's main product is a disposable adhesive patch that monitors body temperature, transmitting data wirelessly and integrating it into hospital monitoring systems or outpatient mobile devices. Blue Spark primarily serves the healthcare industry. Blue Spark was formerly known as Thin Battery Technologies. It was founded in 2003 and is based in Westlake, Ohio.
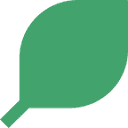
Northvolt focuses on the battery industry. The company's main offerings include the manufacturing of batteries with a lower carbon footprint, as well as the recycling of used batteries into raw materials for new ones. Northvolt primarily sells to sectors such as the automotive industry, construction, and grid systems. It was founded in 2016 and is based in Stockholm, Sweden.
Via Separations is a company focused on energy efficiency in the industrial sector. The company offers innovative filtration solutions that aim to increase process efficiency and reduce costs, specifically by eliminating 90% of the energy currently used in thermal separations. Via Separations primarily serves the industrial sector. It was founded in 2016 and is based in Watertown, Massachusetts.
Loading...